Quantum Computing and Information Processing
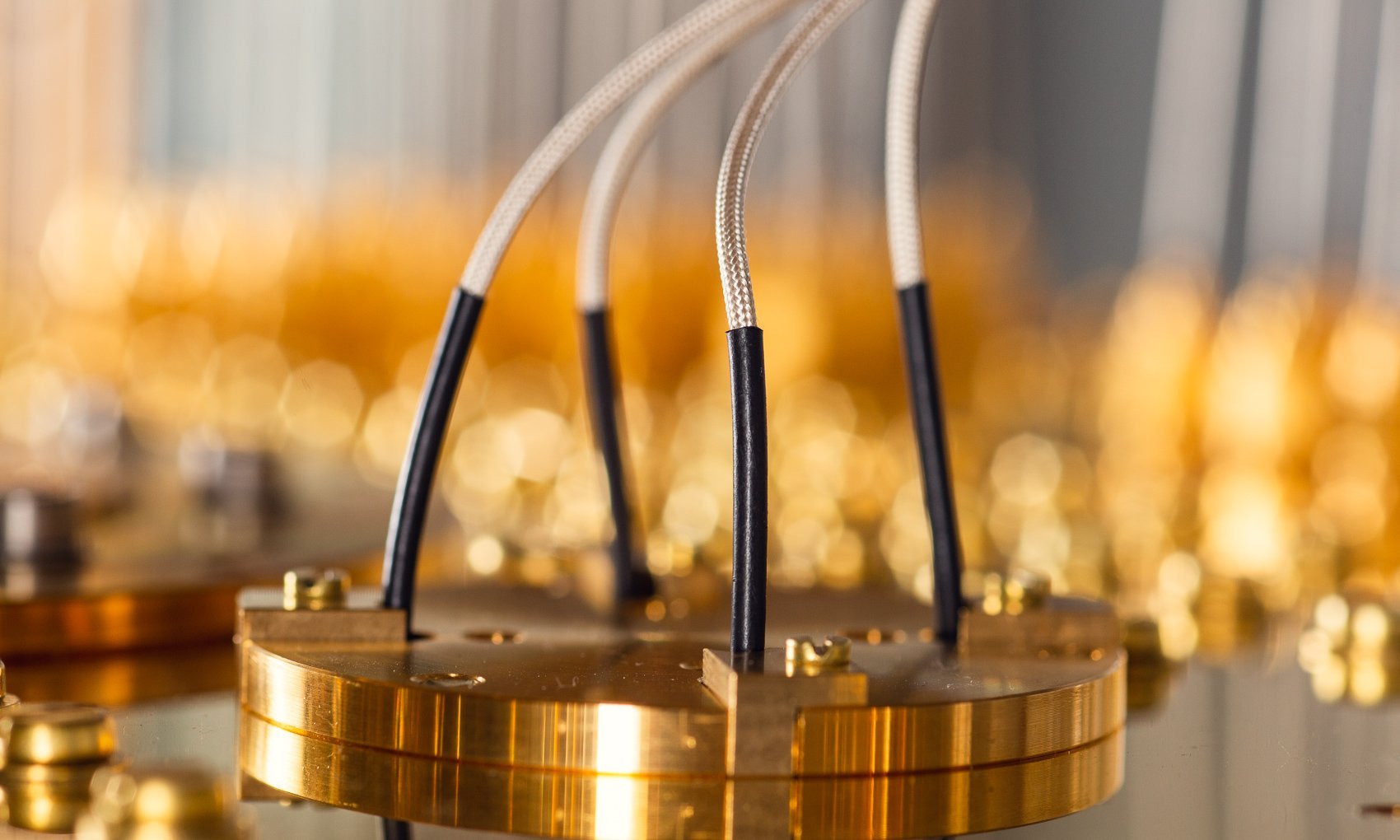
Technological progress goes hand in hand with incessant advances in computing power. Modern personal electronic devices have the computational power of a supercomputer from just a decade ago. Computer-aided designs, logistics, data analysis and cognitive computing have become an essential part of modern life. However, current progress in down-scaling transistors reaches physical limits when approaching atomic scales, and heat dissipation becomes a severe issue with increasing transistor densities.
Above all, certain complex physical problems such as computing energy spectra, correlations or time dynamics in molecular and condensed matter systems are beyond the reach of classical computers. Because of the exponential growth of Hilbert space with the number of particles, such computations require exponential resources preventing the computation of realistic systems.
A quantum computer may provide the means to compute ground-state energies, energy spectra, time dynamics and correlations of such systems efficiently. It is even expected that certain types of optimization problems with application in logistics, time-scheduling and others could be solved more efficiently with the help of quantum effects.
In our experiments, we use superconducting qubits, which can be manipulated on short time scales with respect to their coherence times within a cryogenic environment. Thanks to the relatively simple and reliable fabrication there exists a clear path towards a scalable architecture to realize the building blocks of a future quantum computer.
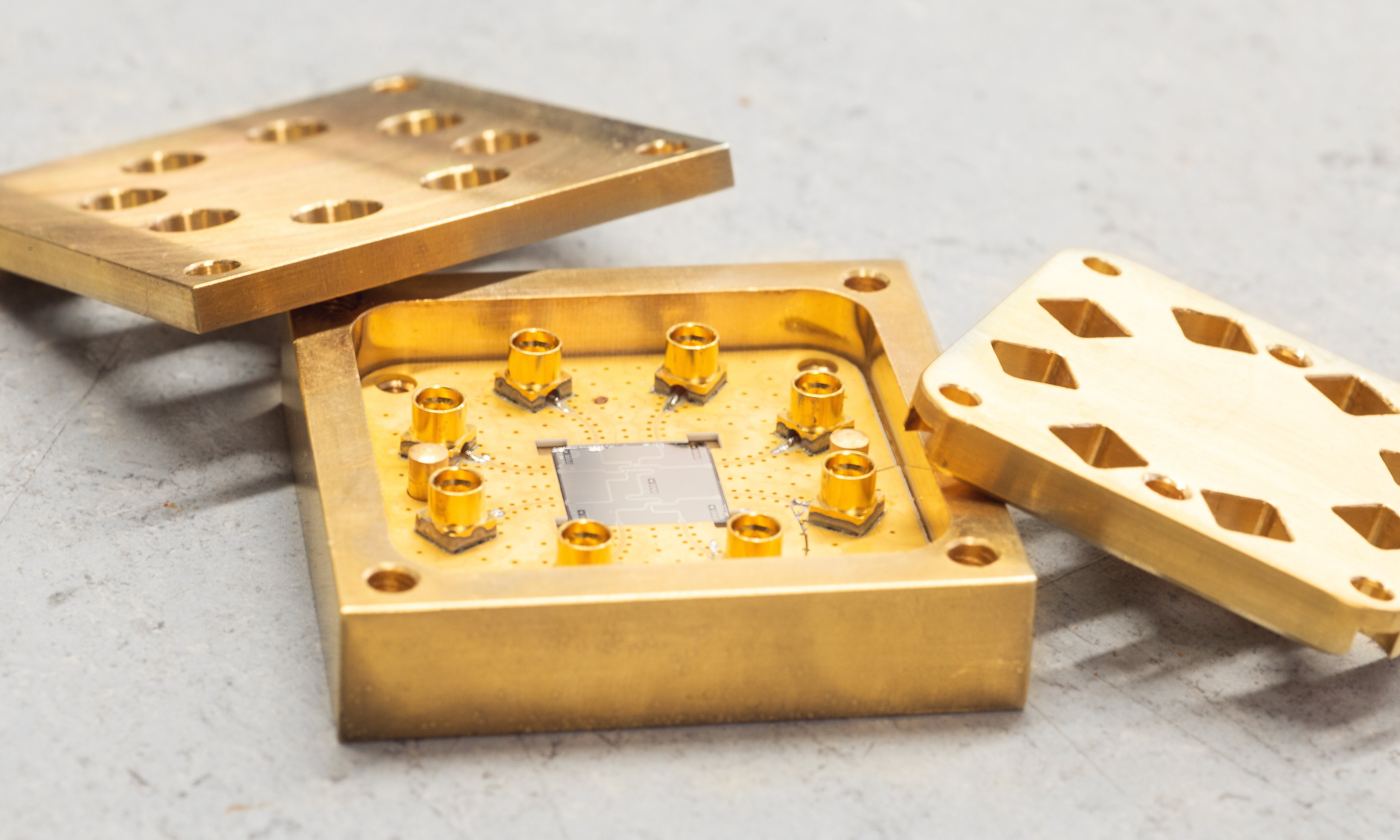
The realization of a scalable quantum computer relies on the continuous improvement of materials, fabrication processes and designs of superconducting qubits and couplers. A further aim is to expand the control capabilities to realize fast and robust high-precision gate operations on single and multiple qubits so that we can eventually offer a reliable platform for testing both algorithms and hardware components. This goal will be reached by focussing on the one hand on scalablity and integration and on the other hand on enabling and exploratory technologies such as novel types of quantum circuits and interactions.
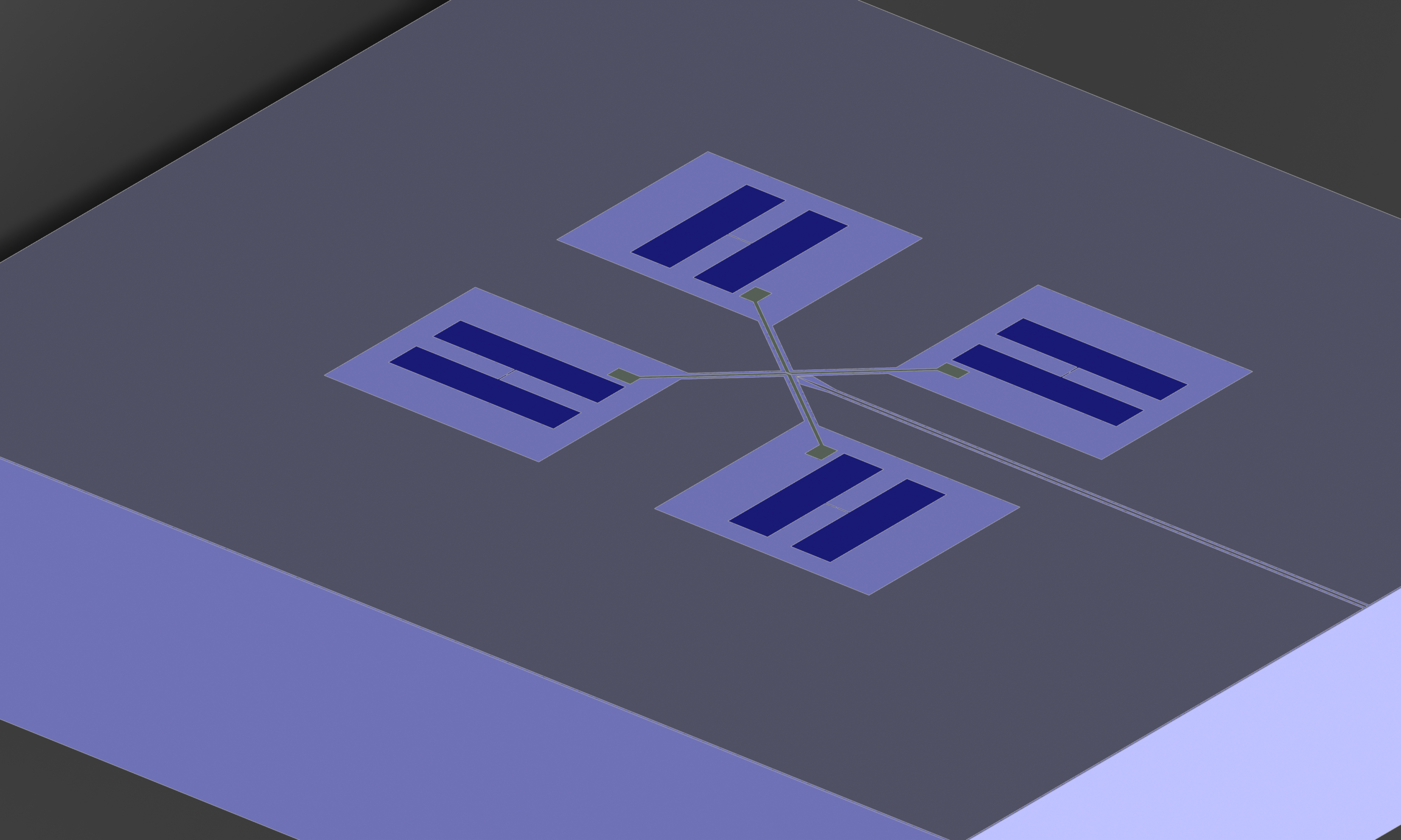
The goal is to develop superconducting qubit architectures and control methods to efficiently generate multi-qubit entangled states. One specific direction is to use couplers connecting multiple superconducting qubits and investigate multi-qubit operations that allow us to entangle and read-out multiple qubits at the same time. We will address the question if there is an advantage in using multi-qubit gates over traditional two-qubit gates in practical experiments by assessing the efficiency of such gates in specific algorithms, e.g., for quantum chemistry. Moreover, we investigate different types of qubits and couplers that allow for fast and high-fidelity gate operations. The devices and methods developed here may enhance the scalability of superconducting qubit platforms and the efficiency of quantum algorithms.
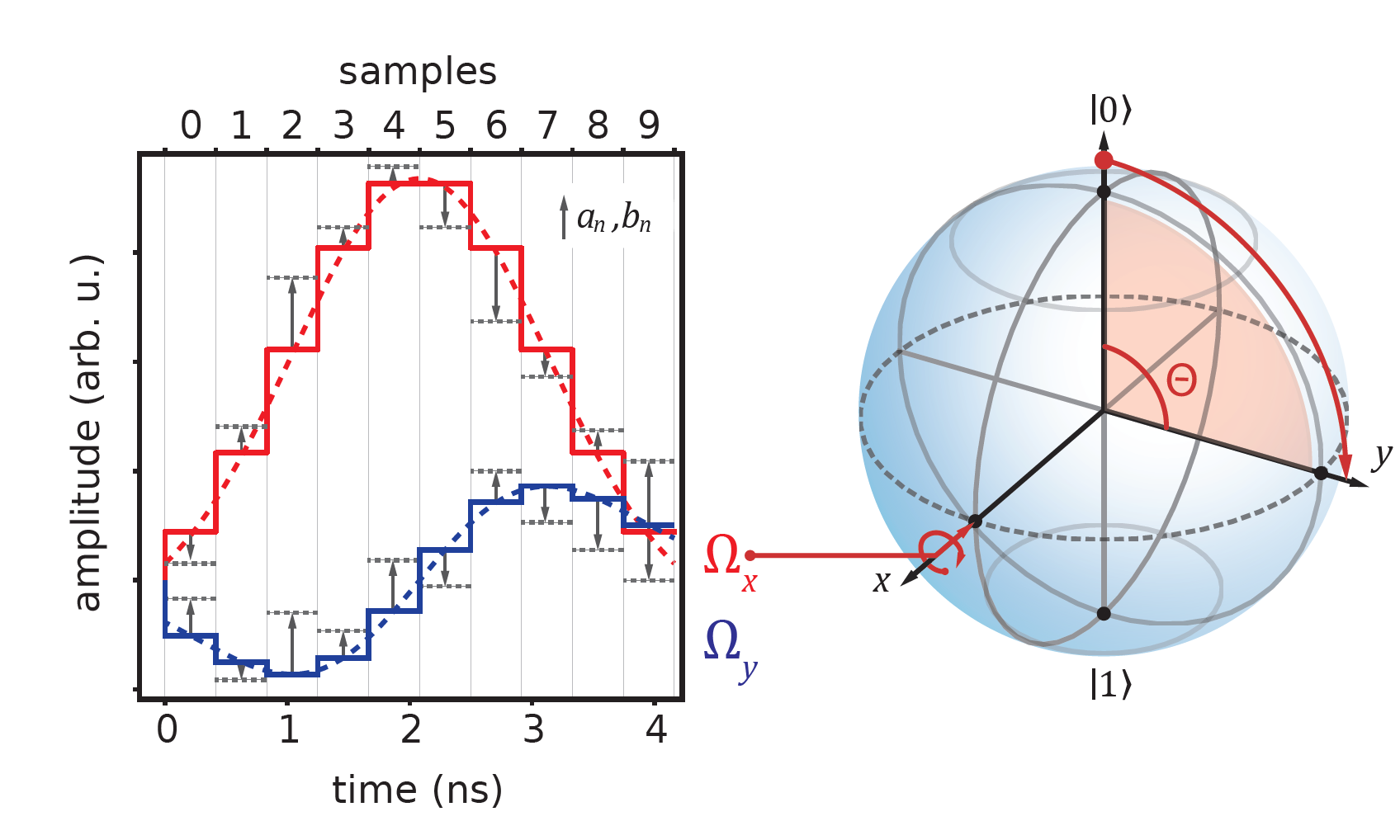
The primary focus of this line of research is on the design, optimal characterization and control of multi-qubit superconducting devices in a circuit QED architecture. We use closed-loop measurements to optimize the tune-up of the system and to obtain high-fidelity quantum gates. Moreover, we address the question how to tailor control and measurements of a complex multi-qubit quantum processor in order to obtain targeted information in the most efficient and robust way. We study experimental techniques to optimize gate operations at the pulse level. Combining these with advanced calibration and characterization methods will allow us to prepare quantum states and run algorithms with high fidelity.
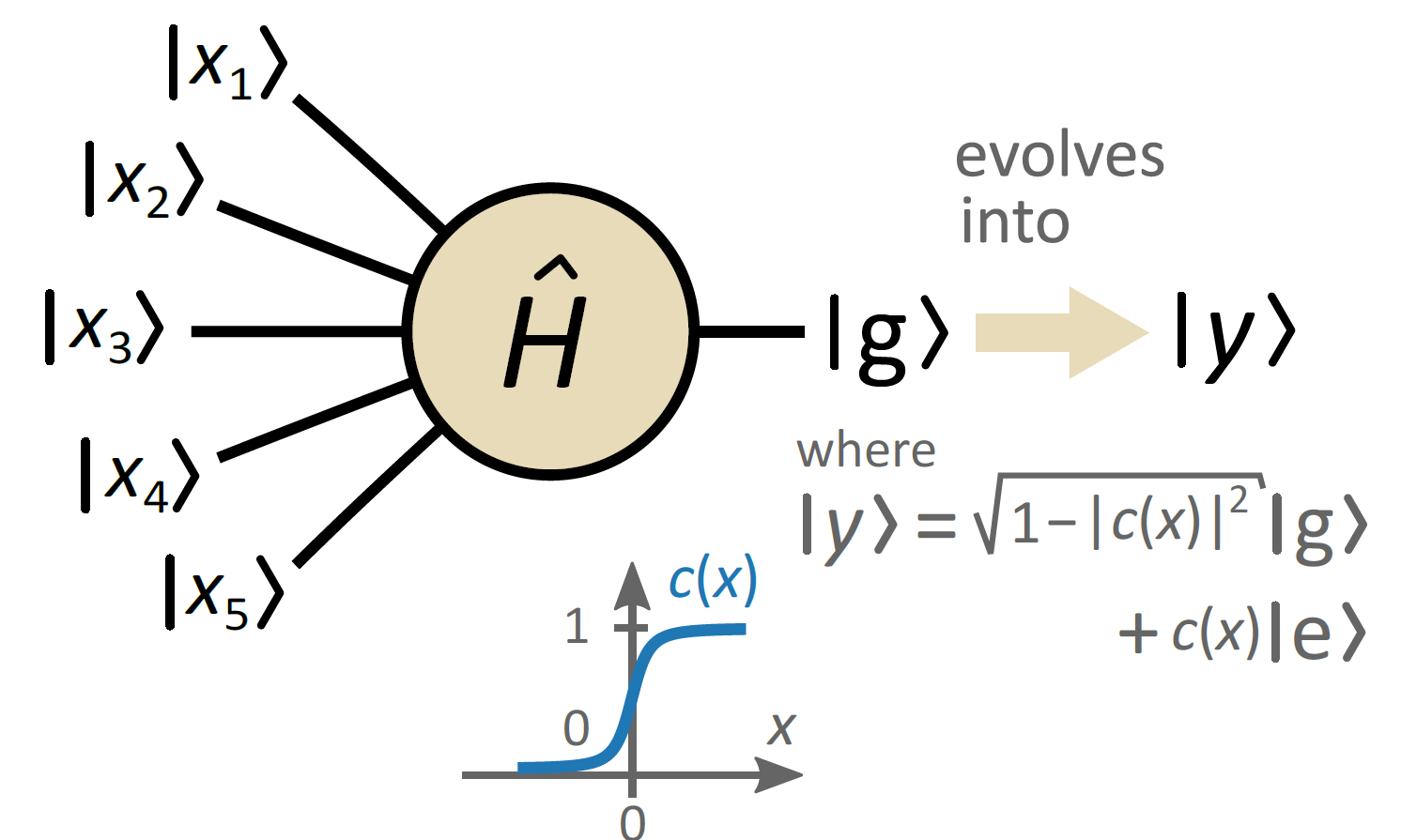
A specific way to realize quantum neuron networks is based on adiabatic ramp quantum neurons. These are implemented via tunable ZZ-type couplings together with an adiabatically varying driving field. Along with higher connectivity brought by multi-qubit couplers we aim to realize first feed-forward networks and explore its capabilities beyond classical approximation.
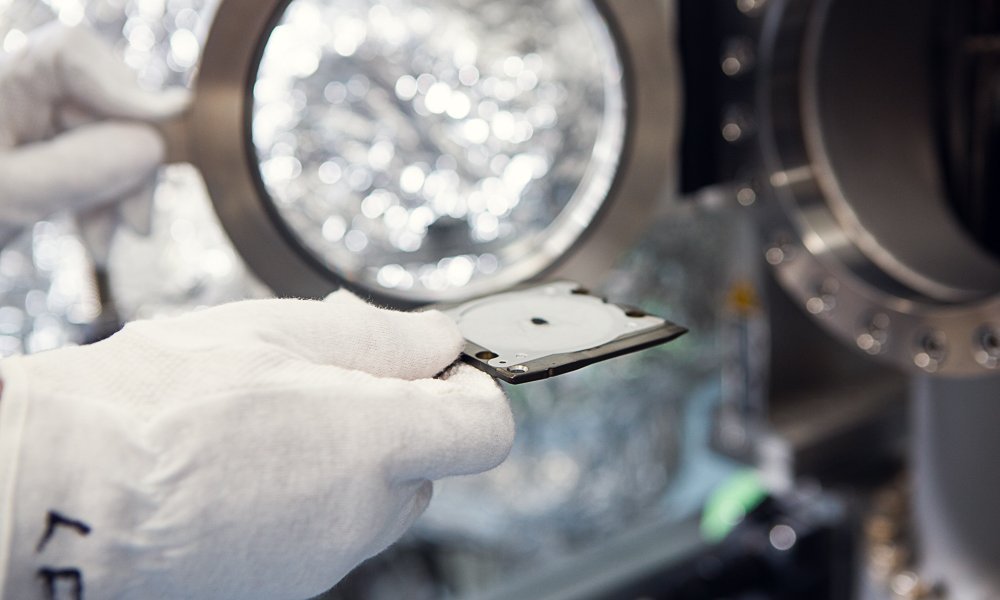
The quality factor of superconducting quantum circuits is determined both by their design and the quality of the materials and interfaces. We explore different materials including high kinetic inductance superconducting thin films to increase the lifetime of qubits. By modelling the effect of interfaces we design qubits which are less affected by surface residues. We also explore different types of qubits that by design feature long coherence times and high anharmonicity.